Discover the history of molecular biology, from groundbreaking milestones to innovations that revolutionized science and medicine. Join our journey!
I. Introduction
A. Definition of molecular biology
B. Importance and relevance of the field
II. Early discoveries and milestones
A. 19th century: Gregor Mendel's work on genetics
B. 20th century: DNA structure and the role of nucleic acids
1. Frederick Griffith's experiments
2. Oswald Avery's identification of DNA as the transforming principle
3. Erwin Chargaff's rules
4. Rosalind Franklin's X-ray diffraction studies
5. Watson and Crick's discovery of the DNA double helix
6. The central dogma of molecular biology: DNA -> RNA -> Protein
III. Development of molecular biology techniques
A. Restriction enzymes and DNA cloning
B. Polymerase Chain Reaction (PCR) and gene amplification
C. DNA sequencing and the Human Genome Project
D. RNA interference and gene silencing
IV. Advances in molecular biology research
A. Gene regulation and epigenetics
B. Post-transcriptional and post-translational modifications
C. Non-coding RNA and its functions
D. Genomics, transcriptomics, and proteomics
V. Molecular biology in medicine and biotechnology
A. Gene therapy and personalized medicine
B. Stem cells and regenerative medicine
C. CRISPR-Cas9 and genome editing
D. Synthetic biology and the creation of artificial life
VI. Current challenges and future directions
A. Ethical considerations and public opinion
B. Emerging technologies and their potential impact
C. Interdisciplinary collaborations and the future of molecular biology
VII. Conclusion
A. Recap of the history of molecular biology
B. Importance of continued research and innovation in the field
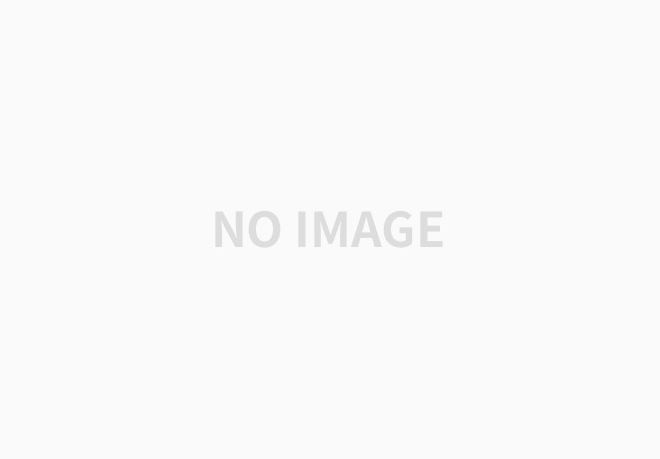
I. Introduction
A. Definition of molecular biology
Molecular biology is a branch of biology that focuses on the study of biomolecules, their functions, and interactions within living organisms. It investigates the molecular mechanisms that govern various biological processes, including gene expression, DNA replication, RNA synthesis, protein synthesis, and cellular signaling. By understanding these molecular mechanisms, molecular biology seeks to uncover the fundamental principles that govern life at the molecular level, ultimately providing insights into various aspects of genetics, biochemistry, and cell biology.
B. Importance and relevance of the field
The importance and relevance of molecular biology are vast, as it has greatly contributed to our understanding of life and various biological processes. Here are some key aspects highlighting its significance:
- Basic understanding of life: Molecular biology has allowed us to comprehend life at its most fundamental level, revealing the molecular basis of cellular processes, such as gene expression, DNA replication, and protein synthesis.
- Medicine: Molecular biology has led to significant advancements in medical research, paving the way for personalized medicine, gene therapy, and targeted treatments for various diseases, including cancer and genetic disorders.
- Biotechnology: The knowledge gained from molecular biology has enabled the development of biotechnological applications, such as genetic engineering, recombinant DNA technology, and CRISPR-Cas9 gene editing, which have revolutionized agriculture, industry, and environmental management.
- Interdisciplinary connections: Molecular biology has fostered collaborations among various fields, including biochemistry, genetics, cell biology, and computational biology, leading to a more integrated understanding of life and its complexity.
- Evolutionary biology: Molecular biology has played a crucial role in studying the evolutionary relationships among organisms, helping us to better understand the origin and diversification of life on Earth.
Overall, molecular biology is of immense importance, as it has not only deepened our understanding of life but also catalyzed advancements in diverse areas, such as medicine, biotechnology, and evolutionary biology.
II. Early discoveries and milestones
A. 19th century: Gregor Mendel's work on genetics
In the 19th century, Gregor Mendel, an Austrian monk, and scientist, laid the foundation for modern genetics through his groundbreaking work on inheritance patterns in pea plants. Between 1856 and 1863, Mendel conducted experiments with over 29,000 pea plants, meticulously observing their traits and how they were passed on to subsequent generations.
Mendel's work led to the formulation of two fundamental principles of inheritance: the Law of Segregation and the Law of Independent Assortment. The Law of Segregation states that each organism inherits two copies of each gene (one from each parent) and passes on only one of those copies to its offspring. The Law of Independent Assortment states that the inheritance of one trait is independent of the inheritance of other traits, assuming the traits are controlled by genes on separate chromosomes.
At the time, Mendel's work was not widely recognized or understood. It was only in 1900, when three scientists—Hugo de Vries, Carl Correns, and Erich von Tschermak—rediscovered Mendel's findings independently, that his work gained prominence and became the cornerstone of modern genetics. This reestablished Mendel as the "Father of Genetics" and marked the beginning of a new era in the understanding of inheritance and the role of genes in determining traits.
B. 20th century: DNA structure and the role of nucleic acids
In the 20th century, major advancements were made in understanding the structure of DNA and the role of nucleic acids in carrying genetic information. These discoveries revolutionized the field of molecular biology and paved the way for subsequent breakthroughs.
1. Frederick Griffith's experiments (1928)
Griffith's work on Streptococcus pneumoniae bacteria demonstrated the existence of a "transforming principle" that could transfer genetic information between bacterial cells. This laid the groundwork for the identification of DNA as the molecule responsible for genetic inheritance.
2. Oswald Avery's identification of DNA as the transforming principle (1994)
Avery, along with his colleagues Colin MacLeod and Maclyn McCarty, discovered that the "transforming principle" was, in fact, DNA. Their experiments showed that purified DNA from one strain of bacteria could transfer genetic traits to another strain, proving that DNA was the carrier of genetic information.
3. Erwin Chargaff's rules (1950)
Chargaff observed that in DNA, the amount of adenine (A) is equal to the amount of thymine (T), and the amount of cytosine (C) is equal to the amount of guanine (G). This finding, known as Chargaff's rules, was crucial in understanding the complementary nature of DNA strands.
4. Rosalind Franklin's X-ray diffraction studies (early 1950s)
Franklin's work on X-ray crystallography provided critical evidence for the helical structure of DNA. Her famous "Photo 51" revealed key details about the molecule's structure, which greatly influenced the subsequent work of Watson and Crick.
5. Watson and Crick's discovery of the DNA double helix (1953)
James Watson and Francis Crick, building on the work of Chargaff, Franklin, and Linus Pauling, proposed the iconic double-helix model of DNA. This model explained how DNA can replicate itself and how genetic information is stored and transmitted.
6. The central dogma of molecular biology (1958): DNA -> RNA -> Protein
Francis Crick proposed the central dogma, which states that genetic information flows from DNA to RNA to proteins. This fundamental concept underpins our understanding of gene expression and protein synthesis.
These pivotal discoveries of the 20th century established DNA as the molecule responsible for storing and transmitting genetic information, provided a comprehensive understanding of its structure, and unraveled the molecular mechanisms governing gene expression and protein synthesis.
III. Development of molecular biology techniques
A. Restriction enzymes and DNA cloning
Restriction enzymes, also known as restriction endonucleases, are proteins that recognize and cleave specific DNA sequences, generating DNA fragments with defined ends. These enzymes are naturally produced by bacteria as a defense mechanism against viruses called bacteriophages. The discovery of restriction enzymes in the 1960s and 1970s revolutionized molecular biology, as they became essential tools for manipulating DNA in the laboratory.
DNA cloning is a technique that involves the production of identical copies of a DNA molecule or a specific gene. The process generally involves four steps:
- DNA isolation: The target DNA fragment or gene of interest is isolated from the source organism.
- Vector preparation: A carrier DNA molecule, known as a vector (often a plasmid), is prepared by cutting it with the same restriction enzyme used to isolate the target DNA. This generates complementary "sticky ends" in both the target DNA and the vector, allowing them to join together.
- Ligation: The target DNA fragment is inserted into the prepared vector using the enzyme DNA ligase, which covalently links the DNA molecules together.
- Transformation and amplification: The recombinant vector containing the target DNA is introduced into a host organism, usually a bacterium, which is then cultured under selective conditions. As the host cells divide and grow, they produce multiple copies of the recombinant vector and the target DNA, effectively "cloning" the DNA fragment.
The development of restriction enzymes and DNA cloning techniques has had a profound impact on molecular biology, enabling scientists to study genes and their functions, produce large quantities of specific DNA sequences or proteins, and generate genetically modified organisms for various applications, such as agriculture, biotechnology, and medicine.
B. Polymerase Chain Reaction (PCR) and gene amplification
Polymerase Chain Reaction (PCR) is a revolutionary molecular biology technique developed by Kary Mullis in 1983, which allows for the rapid amplification of specific DNA sequences. PCR has become an indispensable tool in various fields, such as genetics, clinical diagnostics, forensic science, and molecular biology research.
PCR involves three main steps, repeated over multiple cycles to exponentially amplify the target DNA sequence:
- Denaturation: The DNA sample is heated to separate the double-stranded DNA into two single strands, providing templates for amplification.
- Annealing: The temperature is lowered to allow short, complementary DNA primers to bind to the flanking regions of the target DNA sequence on each template strand. These primers are custom-designed to be specific for the DNA sequence of interest.
- Extension: The temperature is raised slightly to activate a heat-stable DNA polymerase enzyme (usually Taq polymerase), which synthesizes new DNA strands complementary to the template strands, starting from the primers.
After 20-30 cycles, the PCR process can generate millions to billions of copies of the target DNA sequence, enabling its detection and analysis even when the initial sample contains only trace amounts of the target sequence.
Gene amplification using PCR has significantly impacted molecular biology and related fields. Some key applications include:
- Gene cloning: PCR allows for the efficient and rapid isolation of specific DNA sequences for cloning purposes.
- DNA sequencing: PCR-amplified DNA fragments can be directly sequenced or used in next-generation sequencing technologies.
- Diagnostics: PCR is widely used for the detection of viral and bacterial infections, genetic disorders, and cancer biomarkers.
- Forensic science: PCR enables the analysis of extremely small or degraded DNA samples, such as those found at crime scenes, for human identification and paternity testing.
- Gene expression analysis: Quantitative PCR (qPCR) enables the measurement of gene expression levels by quantifying the amount of amplified DNA.
In summary, PCR and gene amplification have revolutionized molecular biology, providing a powerful and versatile tool for studying genes and their functions, as well as for diagnostic and forensic applications.
C. DNA sequencing and the Human Genome Project
DNA sequencing is the process of determining the precise order of nucleotides (adenine, guanine, cytosine, and thymine) within a DNA molecule. The development of DNA sequencing techniques has been crucial for advancing our understanding of genetics, molecular biology, and evolution.
The first widely used DNA sequencing method was developed by Frederick Sanger in the 1970s, known as Sanger sequencing or the chain-termination method. This method uses modified nucleotides that terminate DNA synthesis, generating a series of DNA fragments of different lengths. The fragments are then separated by electrophoresis, and the DNA sequence is deduced from the pattern of fragment lengths.
In recent years, next-generation sequencing (NGS) technologies have emerged, enabling massively parallel DNA sequencing with much higher throughput and lower cost than traditional Sanger sequencing. NGS technologies have significantly accelerated genomic research and enabled the sequencing of entire genomes for many organisms, including humans.
The Human Genome Project (HGP) was a landmark international scientific project aimed at determining the complete DNA sequence of the human genome. Launched in 1990 and completed in 2003, the HGP involved a collaborative effort among researchers from around the world.
The HGP achieved several important goals:
- Sequencing the entire human genome: The project successfully determined the sequence of approximately 3 billion base pairs that make up the human genome, revealing the precise order of the nucleotides and the organization of the estimated 20,000-25,000 protein-coding genes.
- Mapping and annotating the human genome: The HGP generated detailed maps of the human genome, providing valuable information on the location and organization of genes, as well as other functional elements and regulatory regions.
- Developing new technologies: The HGP spurred the development of new sequencing technologies, bioinformatics tools, and data analysis methods, which have been widely adopted in various fields of genomics research.
- Studying human genetic variation: The HGP laid the foundation for projects like the International HapMap Project and the 1000 Genomes Project, which aimed to catalog human genetic variation and better understand the relationship between genetic differences and human health.
The Human Genome Project has had a transformative impact on biology and medicine, providing a wealth of information on human genes, their functions, and their roles in health and disease. The insights gained from the HGP have led to advances in diagnostic testing, personalized medicine, and our understanding of the genetic basis of complex diseases.
D. RNA interference and gene silencing
RNA interference (RNAi) is a natural cellular mechanism for gene regulation and defense against viruses that has been harnessed as a powerful tool for gene silencing in molecular biology research. RNAi was first discovered in the 1990s by Andrew Fire and Craig Mello, who were awarded the Nobel Prize in Physiology or Medicine in 2006 for their pioneering work.
RNAi is triggered by small RNA molecules, including small interfering RNAs (siRNAs) and microRNAs (miRNAs), which are typically 21-23 nucleotides long. These small RNA molecules guide an RNA-induced silencing complex (RISC) to complementary sequences in target messenger RNA (mRNA) molecules, leading to either the degradation of the target mRNA or the inhibition of its translation into protein. The end result is reduced expression or complete silencing of the target gene.
The discovery of RNAi has had a profound impact on molecular biology and biomedical research, enabling scientists to study gene function and regulation with unprecedented precision. Some key applications of RNAi include:
- Functional genomics: RNAi enables researchers to study the function of specific genes by silencing them and observing the resulting phenotypic changes in cells or organisms.
- Target validation: RNAi can be used to validate the role of specific genes in disease processes or as potential therapeutic targets by assessing the effects of gene silencing on cellular or physiological functions.
- Therapeutics: RNAi-based therapies are being developed for various diseases, including viral infections, cancer, and genetic disorders. These therapies aim to selectively silence disease-causing or disease-associated genes, providing a targeted approach to treatment.
- Antiviral defense: RNAi is a natural antiviral mechanism in plants and invertebrates, and its potential for conferring virus resistance in transgenic plants and animals is being explored.
Despite some challenges, such as the efficient and specific delivery of RNAi molecules to target cells and the avoidance of off-target effects, RNA interference has emerged as a powerful and versatile tool in molecular biology, providing novel insights into gene function and regulation, and holding promise for the development of innovative gene-targeted therapies.
IV. Advances in molecular biology research
A. Gene regulation and epigenetics
Gene regulation refers to the complex set of processes that control the expression of genes in a cell, ensuring that the right genes are expressed at the right time and in the right amounts. This regulation is essential for cellular function, development, and adaptation to changing conditions. Gene regulation can occur at various stages, including transcription (when DNA is copied into RNA), RNA processing, translation (when RNA is translated into proteins), and post-translation (modifications to proteins after they are produced).
Epigenetics is a branch of genetics that focuses on heritable changes in gene expression that do not involve alterations to the DNA sequence itself. Epigenetic modifications can influence gene expression by altering the accessibility of DNA to the transcription machinery, thereby turning genes "on" or "off" without changing their sequences.
Key epigenetic mechanisms include:
- DNA methylation: The addition of a methyl group to cytosine bases in DNA, typically at CpG sites, can lead to the repression of gene transcription. DNA methylation is crucial for processes such as genomic imprinting, X-chromosome inactivation, and silencing of transposable elements.
- Histone modifications: Histones are proteins around which DNA is wrapped, forming nucleosomes and higher-order chromatin structures. Post-translational modifications to histones, such as acetylation, methylation, and phosphorylation, can alter chromatin structure and impact gene expression. Histone modifications can lead to either activation or repression of transcription, depending on the specific modification and the histone residue being modified.
- Non-coding RNA molecules: Non-coding RNAs, including microRNAs (miRNAs) and long non-coding RNAs (lncRNAs), can regulate gene expression by interacting with DNA, RNA, or proteins to modulate transcription, RNA processing, or translation.
Epigenetic regulation plays a vital role in many biological processes, such as cellular differentiation, development, and response to environmental stimuli. Epigenetic changes have also been implicated in various diseases, including cancer, neurological disorders, and cardiovascular diseases. Understanding epigenetic mechanisms can provide valuable insights into gene regulation, cellular function, and disease processes, as well as inform the development of novel therapeutic strategies targeting epigenetic modifications.
B. Post-transcriptional and post-translational modifications
Post-transcriptional and post-translational modifications are essential processes that fine-tune gene expression and protein function. They occur after the initial transcription of DNA into RNA or the translation of RNA into proteins, respectively, and involve the addition, removal, or alteration of specific chemical groups or structures.
Post-transcriptional modifications:
- RNA splicing: Introns (non-coding regions) are removed from precursor mRNA (pre-mRNA) and exons (coding regions) are joined together to form the mature mRNA molecule. This process can produce multiple mRNA variants from a single gene through alternative splicing.
- 5' capping: A 7-methylguanosine cap is added to the 5' end of the mRNA molecule, which protects the mRNA from degradation and promotes translation initiation.
- 3' polyadenylation: A poly(A) tail, consisting of multiple adenine nucleotides, is added to the 3' end of the mRNA molecule. This tail enhances mRNA stability and translation efficiency.
- RNA editing: Specific nucleotides within the mRNA sequence can be modified, inserted, or deleted, resulting in changes to the encoded protein sequence. This process expands the diversity of proteins that can be generated from a single gene.
- RNA stability and degradation: Regulatory mechanisms control the stability and degradation of mRNA molecules, influencing their expression levels and translation rates.
Post-translational modifications:
- Phosphorylation: The addition of a phosphate group to specific amino acid residues (typically serine, threonine, or tyrosine) can alter protein activity, stability, or interactions with other proteins.
- Glycosylation: The attachment of carbohydrate moieties to specific amino acid residues can influence protein folding, stability, activity, and cellular localization.
- Ubiquitination: The covalent attachment of ubiquitin molecules to specific lysine residues can target proteins for degradation by the proteasome, regulate protein activity, or modulate protein-protein interactions.
- Acetylation: The addition of an acetyl group to lysine residues can impact protein activity, stability, and interactions with other molecules.
- Proteolytic cleavage: Proteins can be processed by proteases, which remove specific amino acids or peptide fragments, often to activate or inactivate the protein.
- Disulfide bond formation: The formation of covalent disulfide bonds between cysteine residues can stabilize the three-dimensional structure of proteins and modulate their function.
Post-transcriptional and post-translational modifications play critical roles in regulating gene expression and protein function, ensuring that cellular processes are tightly controlled and responsive to environmental cues. Understanding these modifications is essential for gaining insights into cellular function, development, and disease mechanisms.
C. Non-coding RNA and its functions
Non-coding RNA (ncRNA) refers to RNA molecules that do not code for proteins but instead play various regulatory roles in cells. These RNA molecules have diverse structures, sizes, and functions, contributing to the complexity of gene regulation and cellular processes. Some well-known classes of non-coding RNA and their functions include:
- MicroRNAs (miRNAs): These are small (~22 nucleotides) RNA molecules that regulate gene expression post-transcriptionally. They bind to complementary sequences in target messenger RNAs (mRNAs) and promote mRNA degradation or inhibit translation, leading to reduced protein synthesis. miRNAs play essential roles in development, differentiation, cell proliferation, and apoptosis, and their dysregulation has been implicated in various diseases, including cancer.
- Long non-coding RNAs (lncRNAs): These are RNA molecules longer than 200 nucleotides that do not code for proteins. lncRNAs have diverse functions, including regulation of gene expression at the transcriptional and post-transcriptional levels, chromatin remodeling, genomic imprinting, and X-chromosome inactivation. They can act as scaffolds for the assembly of protein complexes, guides for the recruitment of proteins to specific genomic locations, or decoys that sequester proteins or other RNA molecules. lncRNAs have been implicated in various biological processes and diseases, such as cancer and neurological disorders.
- Small nuclear RNAs (snRNAs): These are small RNA molecules that are components of the spliceosome, a large RNA-protein complex responsible for removing introns from precursor mRNA (pre-mRNA) during RNA splicing. snRNAs play critical roles in the recognition of splice sites and the catalysis of the splicing reaction.
- Small nucleolar RNAs (snoRNAs): These are small RNA molecules that guide the modification of ribosomal RNAs (rRNAs), transfer RNAs (tRNAs), and small nuclear RNAs (snRNAs). SnoRNAs are involved in the processing and maturation of rRNAs, ensuring the proper assembly and function of ribosomes.
- Transfer RNAs (tRNAs): Although tRNAs are not typically classified as non-coding RNAs, they do not code for proteins. Instead, they serve as adaptors during protein synthesis, recognizing specific codons in the mRNA and delivering the corresponding amino acids to the growing polypeptide chain.
- Ribosomal RNAs (rRNAs): These RNA molecules are the main components of ribosomes, along with ribosomal proteins. rRNAs provide the catalytic and structural framework for the ribosome, facilitating the decoding of mRNA and the formation of peptide bonds during protein synthesis.
Non-coding RNAs play crucial roles in the regulation of gene expression and various cellular processes. Their study has significantly expanded our understanding of the complexity of the transcriptome and the mechanisms governing cellular function, development, and disease.
D. Genomics, transcriptomics, and proteomics
Genomics, transcriptomics, and proteomics are branches of molecular biology that focus on the comprehensive analysis of an organism's genome, transcriptome, and proteome, respectively. These large-scale, high-throughput approaches have significantly advanced our understanding of the complexity and dynamics of biological systems.
- Genomics: Genomics refers to the study of an organism's entire DNA sequence, including its genes and non-coding regions. Genomic studies aim to identify, annotate, and compare the complete set of genes (or gene products) within a species or among different species. Advances in sequencing technologies, such as next-generation sequencing (NGS), have facilitated rapid and cost-effective genome sequencing, allowing for the identification of genetic variations, gene functions, and evolutionary relationships.
- Transcriptomics: Transcriptomics focuses on the complete set of RNA molecules (transcripts) produced by the genome under specific conditions or at a particular time. This includes messenger RNAs (mRNAs), non-coding RNAs (ncRNAs), and other regulatory RNAs. Transcriptomic analyses are used to study gene expression patterns, RNA splicing, and regulatory mechanisms in response to various stimuli or during different developmental stages. Techniques such as RNA sequencing (RNA-seq) and microarrays are commonly used to measure the expression levels of thousands of genes simultaneously, providing a global view of the transcriptome.
- Proteomics: Proteomics is the large-scale study of the complete set of proteins (proteome) expressed by an organism under specific conditions or at a particular time. Proteomics aims to identify, quantify, and characterize proteins and their post-translational modifications, as well as study protein interactions, localization, and functions. Since proteins are the primary functional molecules in cells, proteomic analyses provide insights into cellular processes, signaling pathways, and disease mechanisms. Techniques used in proteomics include mass spectrometry (MS), two-dimensional gel electrophoresis (2D-GE), and protein microarrays.
Together, genomics, transcriptomics, and proteomics provide a comprehensive understanding of the molecular mechanisms governing cellular function, development, and disease processes. By integrating data from these different "omics" approaches, researchers can gain a more complete picture of the complex interactions and regulatory networks within biological systems, leading to the development of novel therapeutic strategies and personalized medicine.
V. Molecular biology in medicine and biotechnology
A. Gene therapy and personalized medicine
Gene therapy and personalized medicine are innovative approaches to the treatment and prevention of diseases by tailoring medical interventions to an individual's unique genetic makeup and molecular profile.
- Gene therapy: Gene therapy involves the introduction, removal, or modification of genetic material within a person's cells to treat or prevent disease. Gene therapy can be performed using several methods, such as:
a. Gene replacement: Introducing a healthy copy of a gene to compensate for a mutated or nonfunctional gene, often using viral vectors for delivery.
b. Gene editing: Utilizing techniques like CRISPR-Cas9 to correct mutations directly in the genome, restoring normal gene function.
c. Gene silencing: Using RNA interference (RNAi) or antisense oligonucleotides to suppress the expression of a harmful or overactive gene.
d. Gene addition: Introducing new genes to provide cells with a beneficial function, such as immune cells engineered to recognize and attack cancer cells.
Gene therapy has shown promise in treating various genetic disorders, certain cancers, and viral infections. However, there are still challenges to overcome, including efficient and targeted gene delivery, avoiding immune responses, and ensuring long-term safety.
- Personalized medicine: Personalized medicine, also known as precision medicine, aims to tailor medical treatments to an individual's unique genetic, molecular, and environmental factors. Personalized medicine can improve patient outcomes by:
a. Diagnostics: Identifying genetic markers, such as single nucleotide polymorphisms (SNPs) or gene expression profiles, that can predict disease susceptibility, prognosis, or response to therapy.
b. Pharmacogenomics: Examining how an individual's genetic makeup influences their response to drugs, allowing for the selection of optimal drug regimens and dosages to maximize therapeutic benefits while minimizing adverse effects.
c. Targeted therapies: Developing drugs or interventions that specifically target the molecular drivers of a disease, often identified through genomic or proteomic analyses, leading to more effective and less toxic treatments.
d. Prevention: Using genetic information to identify individuals at higher risk for certain diseases, enabling early intervention or lifestyle modifications to reduce disease risk.
Gene therapy and personalized medicine have the potential to revolutionize healthcare by providing more effective, safer, and tailored treatments for patients. As our understanding of genetics and molecular biology continues to expand, these approaches will become increasingly important in the diagnosis, prevention, and management of a wide range of diseases.
B. Stem cells and regenerative medicine
Stem cells and regenerative medicine are interconnected fields that focus on repairing or replacing damaged tissues and organs by harnessing the unique properties of stem cells to promote healing and restore function.
- Stem cells: Stem cells are undifferentiated cells that have the potential to differentiate into specialized cell types, making them invaluable for regenerative medicine. They also possess the ability to self-renew, allowing them to maintain a constant population within tissues. There are two main types of stem cells:
a. Embryonic stem cells (ESCs): Derived from early-stage embryos, ESCs are pluripotent, meaning they can give rise to all cell types found in the adult body, except for the placenta and umbilical cord.
b. Adult stem cells (ASCs): Also known as somatic stem cells, these are multipotent and can give rise to a limited number of specialized cell types. They are found in various tissues, such as bone marrow, skin, and the nervous system, where they play a crucial role in maintaining tissue homeostasis and repair.
Induced pluripotent stem cells (iPSCs) represent another important stem cell type. They are generated by reprogramming adult somatic cells, such as skin or blood cells, into a pluripotent state, sharing similar properties with ESCs.
- Regenerative medicine: Regenerative medicine aims to restore the structure and function of damaged tissues and organs by stimulating the body's natural healing processes or replacing damaged tissues with healthy ones. Regenerative medicine approaches include:
a. Cell-based therapies: Transplanting stem cells or their progeny into damaged tissues to promote regeneration, such as hematopoietic stem cell transplantation to treat blood disorders or the use of iPSCs-derived cells for the treatment of neurological disorders.
b. Tissue engineering: Combining stem cells, biomaterials, and growth factors to create functional tissues in vitro, which can then be implanted into the body to replace damaged or diseased tissues, such as engineered skin grafts or heart valves.
c. Growth factor therapy: Delivering growth factors, which are proteins that stimulate cell growth, differentiation, and survival, to the site of injury to promote tissue regeneration and repair.
d. Gene editing and gene therapy: Utilizing gene editing technologies, such as CRISPR-Cas9, to correct genetic defects in stem cells, which can then be transplanted back into the patient to restore normal tissue function.
Stem cells and regenerative medicine hold great promise for the treatment of a wide range of diseases and injuries, including degenerative disorders, traumatic injuries, and congenital defects. By harnessing the power of stem cells, regenerative medicine has the potential to revolutionize healthcare and improve the quality of life for millions of people worldwide.
C. CRISPR-Cas9 and genome editing
CRISPR-Cas9 and genome editing refer to a groundbreaking technology that allows scientists to precisely and efficiently edit the DNA of living organisms, revolutionizing molecular biology and offering potential solutions for various diseases, genetic disorders, and agricultural applications.
- CRISPR-Cas9: CRISPR (Clustered Regularly Interspaced Short Palindromic Repeats) and Cas9 (CRISPR-associated protein 9) form the key components of a naturally occurring adaptive immune system in bacteria, which defends against invading viruses. In this system, CRISPR sequences store fragments of viral DNA, while the Cas9 enzyme, a nuclease, cuts and inactivates the viral DNA when it is detected. Researchers have harnessed this system for genome editing in other organisms, including plants, animals, and humans.
- Genome editing: The CRISPR-Cas9 technology works as a molecular "cut and paste" tool for editing DNA. The process involves two main components:
a. Guide RNA (gRNA): A custom-designed RNA molecule that is complementary to the target DNA sequence. The gRNA directs the Cas9 enzyme to the specific site in the genome that needs editing.
b. Cas9 enzyme: A nuclease that cleaves the target DNA at the site specified by the gRNA, creating a double-strand break.
After the Cas9 enzyme creates a double-strand break in the DNA, the cell's natural repair mechanisms are activated. There are two primary repair pathways:
a. Non-homologous end joining (NHEJ): An error-prone repair mechanism that often introduces small insertions or deletions (indels) at the break site, potentially disrupting the target gene and leading to its inactivation.
b. Homology-directed repair (HDR): A precise repair mechanism that uses a donor DNA template to introduce specific modifications, such as correcting a mutation or adding a new gene sequence, at the break site.
- Applications: CRISPR-Cas9 has numerous applications across various fields, including:
a. Medicine: Developing gene therapies for genetic disorders, such as cystic fibrosis, muscular dystrophy, and sickle cell anemia; creating engineered immune cells to target and kill cancer cells; and conducting research on the functional role of specific genes in disease development.
b. Agriculture: Developing crops with improved traits, such as resistance to pests, diseases, or environmental stress; enhancing nutritional content; and increasing yield and productivity.
c. Basic research: Studying gene function and regulation, creating animal models for human diseases, and advancing our understanding of genetics and molecular biology.
CRISPR-Cas9 and genome editing have transformed the field of molecular biology and hold great promise for the future of medicine, agriculture, and research. However, ethical considerations, safety concerns, and potential off-target effects must be addressed as the technology continues to advance and approaches clinical applications.
D. Synthetic biology and the creation of artificial life
Synthetic biology and the creation of artificial life involve the design and construction of novel biological systems and organisms using engineering principles and techniques, pushing the boundaries of what is considered "natural" and opening up new possibilities in biotechnology and medicine.
- Synthetic biology: Synthetic biology is an interdisciplinary field that combines biology, engineering, computer science, and other disciplines to design and create biological systems with novel functions or characteristics. Some of the key approaches in synthetic biology include:
a. DNA synthesis and assembly: Designing and constructing custom DNA sequences encoding new genetic pathways or components, which can be introduced into living cells to produce desired functions, such as the production of biofuels, pharmaceuticals, or other valuable chemicals.
b. Genome editing and engineering: Utilizing tools like CRISPR-Cas9 to make precise modifications to existing genomes or to introduce synthetic genetic circuits that can regulate cellular functions or respond to environmental signals.
c. Biological parts and circuits: Designing and constructing modular genetic "parts" and "circuits" that can be assembled to create complex biological systems with specific functions, such as biosensors or gene expression regulators.
- Creation of artificial life: The ultimate goal of synthetic biology is to create entirely new organisms or forms of artificial life that are designed from scratch to perform specific tasks or functions. Some notable achievements in the creation of artificial life include:
a. Minimal genome organisms: Researchers have created organisms with a minimal set of essential genes, simplifying the genetic makeup and providing a better understanding of the core functions necessary for life. An example is the creation of the first synthetic bacterial cell, Mycoplasma mycoides JCVI-syn1.0, which had a synthesized genome designed by the J. Craig Venter Institute.
b. Artificial cells and protocells: Scientists are working on constructing artificial cells or protocells that mimic some of the fundamental properties of living cells, such as self-assembly, metabolism, and replication. These artificial cells could be used to study the origins of life or as platforms for designing novel therapeutic agents.
c. Xenobiology: This branch of synthetic biology focuses on creating organisms with alternative biochemistries, such as organisms that use synthetic nucleotides or amino acids, expanding the genetic code and potentially enabling the production of new biomolecules with unique properties.
The field of synthetic biology and the creation of artificial life have enormous potential for advancing our understanding of biology, as well as practical applications in medicine, agriculture, and biotechnology. However, ethical, safety, and regulatory concerns must be considered as we continue to explore the possibilities and limitations of creating new forms of life.
VI. Current challenges and future directions
A. Ethical considerations and public opinion
Ethical consideration and public opinion play a critical role in shaping the development and implementation of scientific advances, particularly in fields such as molecular biology, where the potential to manipulate life raises profound questions about our responsibilities and the potential consequences of these technologies.
- Ethical considerations: As we continue to expand our understanding of genetics and molecular biology, it is essential to consider the ethical implications of these advancements. Some key ethical issues to consider include:
a. Safety and unintended consequences: Ensuring that new technologies, such as genome editing and synthetic biology, do not inadvertently cause harm to human health, the environment, or existing ecosystems.
b. Access and equity: Addressing potential disparities in the availability and affordability of new treatments and technologies, ensuring that the benefits are equitably distributed and not limited to those with financial means.
c. Informed consent and privacy: Ensuring that individuals participating in research or receiving treatments based on these technologies are fully informed of the risks and benefits, and that their genetic information is protected from unauthorized access or misuse.
d. Playing God and the sanctity of life: Considering the moral implications of creating artificial life or manipulating the human germline, which could have lasting effects on future generations.
- Public opinion: Public opinion plays a crucial role in shaping the policies and regulations that govern the development and application of new technologies. It is essential to engage with the public to ensure that their views and concerns are considered in the decision-making process. Some factors that influence public opinion include:
a. Trust in science and institutions: Public trust in scientists, regulatory agencies, and the research process can significantly impact the acceptance and adoption of new technologies.
b. Media representation and communication: The portrayal of scientific advances in the media and the communication of complex scientific concepts to the public can influence understanding and opinions about these technologies.
c. Cultural and religious beliefs: Cultural and religious beliefs can shape public opinion on issues such as genetic modification, stem cell research, and the creation of artificial life.
d. Education and awareness: Public understanding of the scientific principles and potential benefits and risks of new technologies can impact the level of support or opposition to their development and implementation.
Addressing ethical considerations and understanding public opinion are crucial for the responsible development and application of advancements in molecular biology. Open dialogue, transparent communication, and effective engagement with various stakeholders, including researchers, policymakers, and the public, can help build trust and ensure that these technologies are used in a manner that respects ethical principles and societal values.
B. Emerging technologies and their potential impact
Emerging technologies and their potential impact:
- Artificial intelligence (AI) and machine learning: AI and machine learning techniques can analyze complex biological data, predict protein structures, and guide drug discovery. These computational approaches will revolutionize our understanding of molecular biology, accelerate research, and facilitate the development of novel therapeutics and diagnostics.
- Single-cell sequencing: Single-cell sequencing technologies enable the analysis of individual cells' genomic, transcriptomic, and epigenomic profiles. This technology can provide insights into cellular heterogeneity, developmental processes, and disease mechanisms, leading to improved diagnostics and personalized treatments.
- Gene editing with CRISPR-Cas9 and base editing: The CRISPR-Cas9 system has revolutionized genome editing, allowing for precise manipulation of DNA sequences. Base editing, a newer approach, enables direct conversion of one DNA base to another without double-strand breaks. These technologies hold promise for treating genetic diseases, engineering improved crops, and understanding gene function.
- Organoids and 3D bioprinting: Organoids, miniature organ-like structures grown in vitro, and 3D bioprinting technologies enable the creation of complex, multicellular structures for studying organ development, disease modeling, and drug testing. These approaches have the potential to reduce animal experimentation and advance regenerative medicine.
- Microbiome research: Advances in sequencing technologies and computational analysis have enabled the study of complex microbial communities within various environments, including the human body. Understanding the microbiome's role in health and disease can lead to novel therapeutics, diagnostics, and preventive strategies.
- Nanotechnology in drug delivery and diagnostics: Nanotechnology enables the design of novel drug delivery systems and diagnostic tools, enhancing the efficacy and safety of therapeutics and enabling early disease detection. Nanoparticles can improve targeted drug delivery, minimize side effects, and facilitate real-time monitoring of treatment response.
- Lab-on-a-chip and point-of-care diagnostics: Miniaturized, portable devices integrating multiple laboratory functions on a single chip can perform rapid, on-site diagnostic testing, enabling early detection and personalized treatments. These devices have the potential to revolutionize healthcare delivery, particularly in resource-limited settings.
- Synthetic biology and the creation of artificial life: Synthetic biology involves designing and constructing novel biological systems or organisms with specific functions. This emerging field has applications in biomanufacturing, environmental monitoring, and the creation of artificial cells or organisms, raising ethical and safety concerns that require careful consideration.
- Multi-omics approaches and systems biology: Integrating data from genomics, transcriptomics, proteomics, and other omics technologies allows for a comprehensive understanding of biological systems. Systems biology combines experimental and computational approaches to study complex interactions within cells and organisms, elucidating regulatory networks and identifying potential therapeutic targets.
The impact of emerging technologies in molecular biology is vast, with the potential to transform medicine, agriculture, industry, and our understanding of life itself. However, these advancements also raise ethical, safety, and regulatory concerns that must be addressed responsibly to ensure the beneficial application of these technologies for society.
C. Interdisciplinary collaborations and the future of molecular biology
Interdisciplinary collaborations and the future of molecular biology are closely intertwined, as the rapid advancements in this field require the integration of diverse scientific disciplines to address complex biological questions and develop innovative applications.
- Interdisciplinary collaborations: Molecular biology has increasingly become a collaborative field, drawing expertise from various scientific areas to drive innovation and discovery. Key disciplines that contribute to molecular biology research include:
a. Bioinformatics and computational biology: Analyzing large-scale genomic, transcriptomic, and proteomic data sets, and developing computational models to understand complex biological systems and regulatory networks.
b. Biophysics and structural biology: Investigating the molecular structures, interactions, and biophysical properties of biological macromolecules, such as proteins and nucleic acids, to gain insights into their functions and mechanisms.
c. Systems biology: Combining experimental and computational approaches to study the complex interactions within biological systems and understand how these interactions give rise to cellular functions and behaviors.
d. Synthetic biology and genetic engineering: Designing and constructing novel biological systems and organisms with specific functions, and applying engineering principles to manipulate biological systems.
e. Nanotechnology and materials science: Developing novel materials and nanoscale tools for investigating and manipulating biological systems at the molecular level.
- The future of molecular biology: Interdisciplinary collaborations will continue to shape the future of molecular biology, enabling breakthrough discoveries and transformative applications. Some promising areas of research and potential future developments include:
a. Personalized medicine: Leveraging individual genetic information to develop targeted therapies, optimize drug dosing, and predict disease risk, enabling more precise and effective medical interventions.
b. Gene editing and gene therapy: Expanding the applications of CRISPR-Cas9 and other genome editing technologies to develop treatments for genetic diseases, engineer immune cells to combat cancer, and address other medical challenges.
c. Artificial intelligence and machine learning: Applying advanced computational techniques to analyze and interpret complex biological data, and guide the design of new therapies and diagnostics.
d. Environmental and agricultural applications: Utilizing advances in molecular biology to develop sustainable solutions for food production, such as engineering crops with improved yield, resistance to pests and diseases, and enhanced nutritional content.
e. Biomanufacturing and industrial biotechnology: Harnessing the power of engineered microorganisms to produce valuable chemicals, biofuels, and materials, reducing our reliance on non-renewable resources and fostering a circular bioeconomy.
Interdisciplinary collaborations will be crucial in addressing the complex challenges and opportunities presented by the continued advancement of molecular biology. By combining the expertise of scientists from diverse backgrounds, we can unlock the full potential of molecular biology to transform medicine, agriculture, industry, and our understanding of life itself.
VII. Conclusion
A. Recap of the history of molecular biology
Recap of the history of molecular biology:
- 19th century: Gregor Mendel's work on genetics laid the foundation for our understanding of inheritance, as he discovered the basic principles of heredity through his experiments on pea plants.
- 20th century: James Watson and Francis Crick, along with Rosalind Franklin and Maurice Wilkins, uncovered the structure of DNA, revealing the double helix and elucidating the role of nucleic acids in storing genetic information.
- Restriction enzymes and DNA cloning: The discovery of restriction enzymes allowed scientists to cut and manipulate DNA, paving the way for recombinant DNA technology and the cloning of genes.
- Polymerase Chain Reaction (PCR) and gene amplification: Kary Mullis developed PCR, a revolutionary technique to amplify specific DNA sequences, enabling the study of genes and their functions.
- DNA sequencing and the Human Genome Project: DNA sequencing technologies were developed, allowing researchers to read the genetic code, culminating in the Human Genome Project, which provided a complete reference of the human genome.
- RNA interference and gene silencing: The discovery of RNA interference provided a powerful tool for studying gene function by silencing specific genes, furthering our understanding of gene regulation.
- Gene regulation and epigenetics: Researchers have uncovered complex mechanisms of gene regulation, including epigenetic modifications that affect gene expression without altering the underlying DNA sequence.
- Post-transcriptional and post-translational modifications: The study of processes that modify RNA and proteins after their synthesis has revealed additional layers of complexity in gene expression and function.
- Non-coding RNA and its functions: The discovery of various non-coding RNA molecules has expanded our understanding of the functional roles of RNA beyond coding for proteins, including gene regulation and genome organization.
- Genomics, transcriptomics, and proteomics: The development of high-throughput technologies has enabled the study of entire genomes, transcriptomes, and proteomes, providing a comprehensive view of biological systems.
- Gene therapy and personalized medicine: Advances in molecular biology have paved the way for gene therapy to treat genetic diseases and the development of personalized medicine based on individual genetic information.
- Stem cells and regenerative medicine: The study of stem cells and their potential to differentiate into various cell types has opened up new avenues for regenerative medicine and tissue engineering.
- CRISPR-Cas9 and genome editing: The development of CRISPR-Cas9 technology has revolutionized genome editing, enabling precise manipulation of DNA sequences with potential applications in medicine, agriculture, and research.
Throughout its history, molecular biology has made remarkable progress in understanding the fundamental principles of life at the molecular level. Interdisciplinary collaborations and continued research hold great promise for future discoveries and transformative applications in various fields.
B. Importance of continued research and innovation in the field
Importance of continued research and innovation in the field:
- Advancing human health: Continued research in molecular biology can lead to the development of novel therapeutics, diagnostics, and personalized medicine approaches, significantly improving the prevention, diagnosis, and treatment of various diseases, including genetic disorders, cancer, and infectious diseases.
- Understanding the basis of life: Molecular biology allows us to delve deeper into the fundamental processes governing life at the molecular level. Continued research will provide new insights into gene function, regulation, and the complexity of cellular processes, enhancing our understanding of how life works.
- Agricultural and environmental applications: Innovations in molecular biology can contribute to the development of sustainable agricultural practices, such as the engineering of crops with improved yield, resistance to pests and diseases, and enhanced nutritional content. Furthermore, molecular biology research can help address environmental challenges, such as developing strategies for bioremediation and biodiversity conservation.
- Industrial and biotechnological applications: Continued research can lead to the development of new biomanufacturing processes and products, harnessing the power of engineered microorganisms to produce valuable chemicals, biofuels, and materials, reducing our reliance on non-renewable resources and promoting a circular bioeconomy.
- Ethical considerations and responsible innovation: Ongoing research is essential to address the ethical, safety, and regulatory concerns associated with the development and application of molecular biology technologies. By understanding the potential risks and benefits, we can ensure responsible innovation that respects ethical principles and societal values.
- Workforce development and economic growth: Continued research and innovation in molecular biology drive the growth of the biotechnology sector, creating jobs, fostering economic development, and enhancing global competitiveness in the life sciences industry.
- Interdisciplinary collaboration: The field of molecular biology benefits from and contributes to the advancement of various scientific disciplines, such as bioinformatics, biophysics, and synthetic biology. Continued research fosters interdisciplinary collaboration and integration, leading to breakthrough discoveries and transformative applications.
- Inspiring the next generation of scientists: Research and innovation in molecular biology not only contribute to scientific knowledge but also inspire the next generation of scientists, fostering curiosity, creativity, and critical thinking skills essential for the future of science and society.
In summary, continued research and innovation in molecular biology are crucial for addressing global challenges in health, agriculture, environment, and industry, while expanding our understanding of the fundamental processes governing life. As we continue to push the boundaries of knowledge, we unlock the potential of molecular biology to transform our world and improve the quality of life for future generations.
댓글